
The Nanotech View of the Microbiome (Kavli Roundtable)
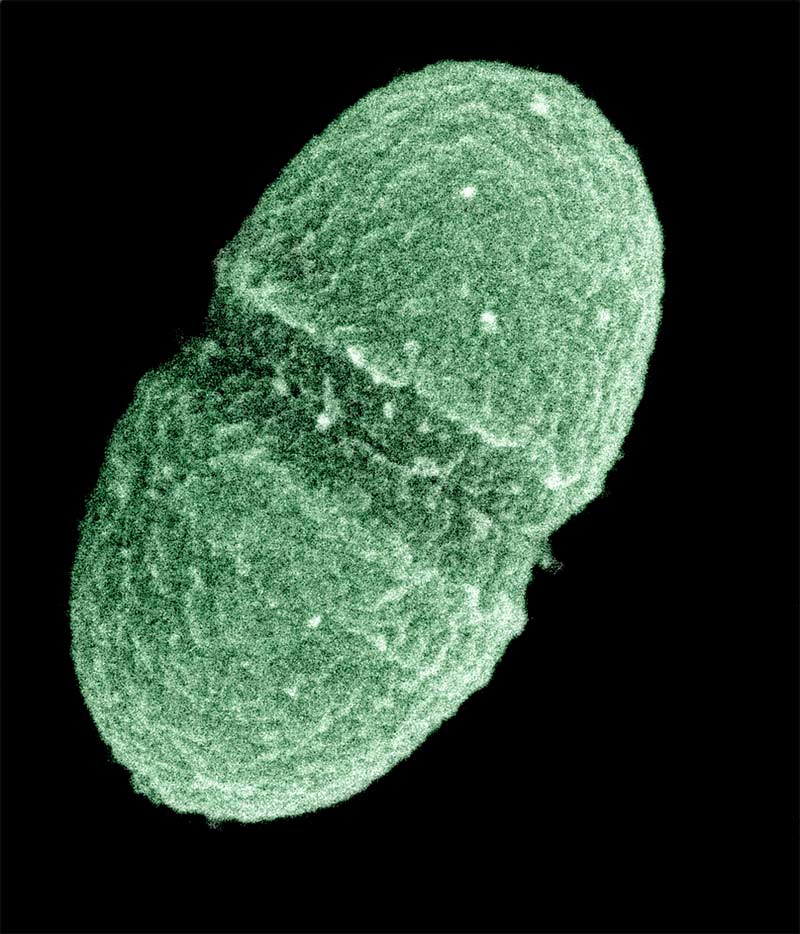
Alan Brown, writer and editor for the Kavli Foundation, edited this roundtable for Live Science's Expert Voices: Op-Ed & Insights.
Microbiomes — communities of microorganisms — exist nearly everywhere, from the soil and the sediment under oceans, rivers and lakes to the landscapes of the human body. They are ubiquitous, mediating the interactions of plants and animals with their environments, and yet we know very little about them.
The Kavli Spotlight, a series of roundtables and live Internet events, has previously covered how the human microbiome influences brain development, and how the study of natural microbiomes drives the search for extraterrestrial life. Our latest roundtable looks at the role of nanoscience and nanotechnology in revealing microbiome communities.
The challenge is significant. Within only a few grams of soil or ocean sediment, rich and complex ecosystems exist that contain hundreds of thousands of different microbial species. Scientists cannot yet grow the vast majority of these single-celled organisms in a lab, and so they are immune to classification by conventional technologies.
Nanoscience may be able to help tease apart how the members of natural microbiomes interact with one another. To discuss this, the Kavli Foundation has invited two leaders in the field:
Eoin Brodie is staff scientist in the Ecology Department at the U.S. Department of Energy (DOE)'s Lawrence Berkeley National Laboratory, and adjunct assistant professor in the Department of Environmental Science, Policy and Management at the University of California, Berkeley. He has pioneered technologies for accurately measuring microbiome community dynamics.
Jack Gilbert is principal investigator in the Biosciences Division at the DOE's Argonne National Laboratory and associate professor in the Department of Ecology and Evolution at the University of Chicago. He has studied the microbiomes that exist within hospitals and is working on ways to use bacteria-embedded nanostructures to rebuild infant microbiomes.
Below is an edited transcript of their discussion. The participants have also been provided the opportunity to amend or edit their remarks.
The Kavli Foundation: What makes a microbiome a microbiome? Is it only about size, or does it require a certain complexity?
Jack A. Gilbert: A microbiome is a community of single-celled microbes. It could include bacteria, fungi, protozoa, algae and viruses. It's a little community whose members are interacting with each other. It can be anything, from ten different species to 1,000 species to 200,000 species.
Eoin Brodie: Consider this analogy: Think of all the different things you might find in a tropical forest. You've got different types of trees and animals and insects. All these things have evolved to work together to form some sort of stable system, in many cases, an ecosystem. So a microbiome is the microbial version of that forest ecosystem. Individually, each different species provides different functions that, together, are essential for the stability and activity of the system. [Body Bugs: 5 Surprising Facts About Your Microbiome Countdown]
TKF: Are there properties that emerge when microbiomes reach a certain size or level of complexity? Are they different from the properties of individual microbes?
J.G.: There are. This is an area of ongoing research, though we can start by looking at how ecological theory plays out in larger organisms. That helps us interpret and predict what microbiomes might do as they grow in complexity.
As complexity increases, we see more interconnections in the system. Think of it like a food web. If it combines multiple insects, trees, plants, and other things, it is potentially more stable than if it has only a single insect and a single tree. The more participants, the more interactions, and these interactions trigger still more interactions. Together, they regulate the abundance of specific types of organisms. Nothing takes over, they all share resources.
At exactly what point an ecosystem becomes stable or resilient is less clear. Macro-ecological theory suggests that when there are more connections, you build in redundancy. This makes the system more robust and resistant to disturbance, though there is a sweet-spot that may be hard to define. Larger ecosystems may have several organisms doing the same thing, though not necessarily at the same time or in the same place. But those organisms could step in when another organism performing that function cannot do so.
J.G.: This is an interesting point. The very definition of a highly robust community or ecosystem is inherent flexibility. It's like a reed bending in a stream, flexing with changes in stress and pressure. Redundancy is part of that. There may be 20 organisms that produce methane, which is then used by other organisms. The members of that methane-producing community will respond differently to changing conditions. One might grow better at higher temperatures, another if temperatures drop. But the fundamental function of that assemblage producing methane, hasn't changed.
TKF: Microbiomes are clearly complex and interconnected. They can have hundreds of thousands of different species. How do we begin to understand something like that? What's the current state of the art?
J.G.: There are multiple states of the art.
E.B.: It's true. For example, we can only grow between 0.001 percent and maybe 10 percent of the microbes we find. For some systems, like the human gut, we are getting better because we know more about them.
In soils, we're not very good. That's because it's very hard to predict what these microbes need to grow. They may have unusual nutritional requirements are, or need other organisms to grow. It almost impossible to grow them in a pure culture.
One window into their function has been things that Jack has pioneered, using metagenomics and sequencing technologies that were developed for human genome sequencing. We can apply those technologies to these incredibly complicated microbial communities.
So we take this community apart, just like an enormous jigsaw puzzle, and break it up into tiny, tiny molecular pieces that we can measure with sequencing machines. The real challenge, however, is putting those pieces back together again in a way that tells you something about the whole community. So, that's one approach.
Another approach involves imaging organisms. You can see them using visible light or other wavelengths, identify their shapes, and learn about the chemistry associated with them. We have done that in some very simple artificial microbial communities we’ve grown in the lab. The challenge is finding ways to apply these technologies to increasingly more complicated systems.
J.G.: You know, you can put "omics" at the end of anything and get a new tool out of it. Genomics measures genes. Transcriptomics covers RNA transcribed from genes. Proteinomics looks at proteins folded by transcribed RNA. Metabolomics analyzes the chemicals and metabolites mediated by those proteins. There's a whole slew of them, and that means we have a lot of tools that can interrogate the components of the system. [The Hunt for Alien Extremophiles is Taking Off (Kavli Q+A) ]
One of our key challenges is to integrate all this information. Eoin's been developing some techniques to attack this problem by compiling this data into an interoperable data framework. It's all very well having a genome, a transcriptome, a metabolome — but pulling those together and creating knowledge out of the chaos can sometimes be an über challenge.
E.B.: I'd say it's one of our grand challenges at the moment, and it is not going to be solved any time soon.
TKF: Why so long? Are we talking weeks, months or years?
J.G.: Decades.
TKF: For a single one?
J.G.: Sometimes. We're developing novel, high-throughput technologies that can help to alleviate that problem. But let's say I have a thousand genes. I don't know what function they encode. We may be able to express a small fraction of them and fold only some of their proteins. I might be able to figure out the function of maybe five of those proteins — I'm being generous. So out of 1,000 targets, today it would be reasonably simple for me to examine five of them. What about the other 99.95? What do we do with those?
E.B.: It's a huge roadblock, but there's a whole new set of high-throughput technologies to automate this process. There are technologies for producing and folding proteins, screening protein function, and finding protein structures without crystallization. All of those things sort of exist, but there's no one lab or initiative that's pulling them together. And that's what we need to understand microbiomes.
J.G.: Even so, it's a big problem. Let me give you an example. E. coli has been our main microbial bacterial workhorse for 100 years, and we still don't know what one-third of the genes do. It's kind of crazy. I work on something called the Microbiome Project, which estimates that there are well over 50 million bacterial species on Earth. We know two-thirds of the genome of one of them.
Still, we can study microbiomes in different contexts. For example, we can look at the emerging properties of an ecosystem, such as its ability to generate methane or consume carbohydrates. Then we can make and test predictions about the functions that community needs. It would be much better if we had all that genomic information, but we don't need it to advance our understanding.
E.B.: Right, this glass really is half full. There are all these amazing chemistries that microbes perform that that can do really wonderful things for humanity, like providing new antibiotics and nutrients for crops. It's pretty much an unlimited resource of novelty and chemistry — if we can develop improved tools to tap into it.
TKF: How does nanoscience help?
E.B.: One of the great advances in sequencing a genome is parallelizing biological assays. So instead of sequencing 12 or 96 or even a few hundred strands of DNA at a time, we can sequence millions at a time. To characterize the biochemistry and chemistry of microbial communities, we have to scale in the same sort of way.
Using nanotechnology, we can build nanofluidic devices to run these assays. These would be the size of semiconductor chips, with nanoscale channels to capture tiny samples of DNA and test them with tiny amounts of reagents, all in parallel. We could use nanoscale imaging sensors to detect these reactions, instead of the large cameras we use today. And we can use semiconductor technologies to make tens of thousands of them from a single silicon wafer, which massively reduces the cost of those assays.
There are clear paths to do this, but we need to rally around the challenge and bring different industries, like the semiconductor industry, together.
J.G.: One of the most exciting things, from my perspective, is to use nanoscience to reduce the complexity of our datasets. Let's say I have 100,000 organisms in a few grams of ocean sediment or soil. I want to understand the role of a complex lipid in this community. If I knew which microbes were involved, I could target them and analyze which genes created or used that lipid. But I don't, so instead, I bind a quantum dot nanoparticle to a food particle used in making the lipid. The organisms that consume it will light up under x-ray analysis.
That will show me the active organism. Then I can start looking for the genes that degrade or transform that lipid. We can use it to narrow down our search window to something that's a little bit more manageable. There are many ways we can deal with this, but this is a good nanotech route.
E.B.: There've been some early successes, but also issues. A quantum dot may be tiny to us, but it is a big thing to a microbe. It can be hard to actually get it inside a cell. The organisms that ingest it in your experimental system may not be the ones that ingest it in nature. Still, variants of those approaches have a lot of potential.
J.G.: As we've always said, my very act of observing this system has changed its nature. Still, either you don't bother or you try these techniques. You've just got to be careful interpreting what you see. Very careful.
E.B.: Science is built on observation, manipulation, more observation, reforming your hypotheses, and repeating that cycle. Manipulation is a key part of that.
Think about how we've manipulated individual microorganisms to understand their function. We start with a hypothesis about a gene's function, knock it out, and see if the organism has lost that function. We can then add that gene back and see if it regains that function, which would prove the hypothesis.
We don't have an analogous way of doing that in a complicated microbial community. We need to knock out an entire species to see if they perform a particular function and observe what happens when that function is not present.
A new approach to genetically engineering individual organisms might help. It's called CRISPR, and it is based on tricking bacteria into self-destructing. For this to work, you have to introduce a genetic construct, a plasmid or something like it, into the bacterial cell. Then it will create a protein that triggers a highly specific self-destruct mechanism. Many bacteria won't take up pieces of foreign DNA for that very reason, because it might mess them up.
Nanoscience might be able to help us trick bacteria into ingesting this plasmid. For example, as Jack was saying, we can stick a quantum dot onto various molecules to identify compounds that bacteria will regularly ingest. We could also attach a CRISPR payload to those same molecules to trigger self-destruction, or knock out or potentially add a new function to the organism. CRISPR and a few other analogous technologies are potentially transformative for microbiome research, and nanotechnology could help us find the delivery mechanisms we need to make it work.
TKF: Is this something we can do relatively soon or are we talking about decades of research?
E.B.: People are doing it now, but they're using E. coli and other organisms that we know and can manipulate in the lab. We've already worked out the theory of how we could target a strain of microbes in a natural microbiome. We know it will work on some bacteria, but it will be difficult to inject these pieces of DNA into others. That's a big research challenge right now.
TKF: What about applying some of the nanotechnology being developed to study the brain to investigate microbiomes in the soil or water?
E.B.: There's amazing work going on in developing miniaturized sensors based on radio frequency identification technology, or RFID. RFID tags are used by companies to track shipments. They can both transmit and acquire energy from radio waves, so they don't need batteries. It gives us a way of getting information from very small sensors without any wiring.
Researchers want to inject them into the brain to sense electrical impulses. I'm not exactly sure how that would work, but the idea is to wind up with a distributed network of sensors. You could read out their location and what they sense remotely.
Now, think about doing something like that in soil. We could make tens of thousands of them from a single silicon wafer, mix them with soil, and plant something. As the roots grow and pass the sensors, we would get a readout of things like temperature, moisture, pH, oxygen concentration, the presence of specific chemicals, and how that initial reading changes over time.
We could build these complicated three-dimensional pictures of how microbes are influencing the area around the root and soil. And perhaps we can use that in an agricultural setting to optimize things like water irrigation and fertilization.
J.G.: We could also use quantum dots here. We could, for example, tag an amino acid with a quantum dot, stick it in the community, and see which members take it up so we can determine who's active.
The cool thing about this technology is that if you take a small sample of soil, maybe 10 microns by 10 microns, you could theoretically use this technique to identify where the active members of that community are. In a community of thousands of organisms, not all will be active at the same time. Some may be living with a very, very low level of activity, waiting for the right conditions to wake up. So we've got to map not only the 3D location of the organisms, but the fourth dimension of time to understand how that community is changing and responding to environmental stimuli.
TKF: You've done something similar with hospital floors, correct?
J.G.: We had a grant to examine the microbiome of hospitals, specifically a new, $800 million facility being built in Chicago. We started looking at the floor when the building was an empty shell and watched as doctors and patients moved in and it became an active, functioning hospital.
We wanted to see how the ecology of that microbiome changed. That might give us some insights into health care-associated infections, the dissemination of antibiotic resistance, and the development of pathogen reservoirs.
It quickly became obvious that the vast majority of the bacteria released by people in the hospital die shortly after landing in what is a remarkably inhospitable ecosystem. We want to understand which ones remain active and which ones go dormant and could revive under different conditions. That's very important to understanding the transmission of diseases in hospitals, and how to control and manipulate microbial ecosystems in our homes, offices and public spaces.
TKF: So what happens next?
J.G.: It's a huge study. We're still working on it. It's an enormous study. We did it every day for 365 days, and generated 8.5 million data points. They included everything from activity assays and bacterial cultures and DNA sequencing to patient and staff medical records. We are teasing apart this complex database of interactions to see how this system actually developed and how it works.
We would like to continue that monitoring. We would like to use some of these novel sensor technologies to continuously monitor this ecosystem and generate this data in a regular, detailed fashion. High-frequency spatial and temporal data is incredibly important if we want to discern trends and understand how to manipulate ecosystems.
TKF: How would you use nanotechnology in your hospital project?
J.G.: As Eoin said, it's about shrinking our sensors down to very small scales. In a built environment, especially a hospital, people don't want to see these things. We need to take samples, process and analyze them, and transmit the data in a space smaller than a light switch. So we need to make everything incredibly small. That means immobilizing probes or primers on certain nanomaterial surfaces and using nanofluidics to reduce the amount of samples we need to capture.
We would like to go even smaller, and compress these capabilities into a pill that you could swallow so you could analyze the human microbiome — or metabolome or even the proteinome — at any point in the gut. You could even put an RFID transmitter in there, so the pill could communicate with your phone and you could see what your microbiome was doing in real time.
TKF: What other things might nanoscience do? Could it provide information that biologists typically cannot access?
E.B.: You know, the same tools used in nanoscience to analyze materials and processes at the atomic scale are being used to understand microbial processes and microbial communication networks. One good example is electrical conductivity. Some microbes conduct electricity, which is how they make the energy they need to live. These processes are very diverse and varied, and researchers have used atomic force microscopy and similar nanoscience tools to understand how those electrons flow at the atomic scale.
At the same time, researchers are studying how to couple these bacterial nanowires to inorganic or organic nonliving things. These nanowires can transfer electrons over long distances, and have incredible properties that are very different from our man-made wires. We can learn by biology, and we can also fuse biology with our electronics.
TKF: What about using nanoscience to improve agriculture?
E.B.: We typically use chemicals, especially nitrogen in the form of ammonia, as fertilizer. Microbes can also generate nitrogen by taking carbon and using it fix atmospheric nitrogen into ammonia. Some bacteria do this within certain plant roots, but we'd like to look at nitrogen-fixing bacterial that live in other parts of many plants. Nanoscience has a role in understanding how those microbes talk to plants, how they share metabolites, and what regulates nitrogen fixation. If we could do that, we might be able to improve crop productivity and reduce or eliminate fertilizer use.
TKF: What about manipulating the microbiomes in homes or people? Could nanoscience help with that?
J.G.: Eoin was just talking about restructuring the microbial environment for plants. We could do something similar in our buildings to give children the microbial exposure to develop a healthy immune system.
E.B.: Exactly. The early months of life are critical to the development of our immune system. The microbiome in our home may have a big impact on this. For example, if you have two large dogs that go outside, you're less likely to develop asthma. The hygiene hypothesis says this is because you are exposed to a greater diversity of microbes that the dogs bring inside. Cleaning and disinfecting prevents this exposure, and it may contribute to the rise of such inflammatory disorders as asthma and eczema.
J.G.: Exactly. We're interested in constructing new architectural interfaces and environments that give our children the right microbial exposures. That involves working with nanoscale interfaces. After all, a bacterial cell is only 700 or 800 nanometers across, and we're talking about creating nanostructures to understand and manipulate its surfaces.
For example, we're very interested in constructing materials with pockets with embedded nanoparticles. These nanoparticles would have chemically modified interfaces that would attract the right kinds of microbes.
TKF: How would you use these ideal microbiome environments?
J.G.: We might embed nanoparticles in 3D printing materials to promote an environment that enables the stable formation of biofilms of bacteria. We might be able to use them as probiotics that a child could take to reconfigure the microbiome in his or her gut. We might have microbial 3D printed walls or floors or carpets or even chairs or door handles.
We're exploring ways to create very specific kinds of 3D printing inks that promote the development of specific kinds of microbiome. This may sound a little bit bizarre, but there's very hard science underneath it. You can't create a carpet and hope for the best. You have to understand how to appropriately manipulate microbiomes, and then create materials that interact with the right microbes and support a thriving microbiome.
E.B.: That's a really interesting concept. You know, our buildings filter out everything below a certain particle size. Perhaps we could engineer intelligent filters that weed out dangerous toxins but allow more of the outdoor microbiome to enter. That would be an amazing contribution. There's no doubt that the microbiome we've evolved with has to have some impact on our heath, particularly in the early life stages.
TKF: A final question. The use of nanoscience to study the microbiome is so new, I'm not even sure we can call it an emerging field yet. How do we achieve the critical mass of researchers we need to achieve significant breakthroughs?
E.B.: We clearly need to work across disciplines and keep extending our networks of researchers. Jack and I have a certain network, and then there are nanoscience researchers who are thinking about the intersection between their work and biology. We need to keep reaching out.
We also need to keep talking about the potential of the microbiome to improve the health of our planet, the health of humanity, our production of food, and our fundamental understanding of our world. No matter what discipline you're in, I think we're asking compelling questions and posing challenges that people can find scientifically interesting.
So we need to get our questions out there, seed the broader community with some potential ideas of where nanotechnology might fit, and I think people will find ways to use nanoscience in ways we never would have imagined.
J.G.: Our team is working with Argonne National Labs and at the University of Chicago, which have large efforts in nanoscience, to implement some of the concepts we've been talking about.
One of the major things we need to overcome is nomenclature. What I call the surface is not what they call the surface. What I call a biological agent is not what they call a biological agent. We have many words for which we have two separate meanings. Since we don't speak the same language, it is often much harder to get things started.
We also need funding initiatives. When the U.S. National Institutes of Health committed $180 million to the Human Microbiome Project, lots of clinicians jumped at the opportunity. There has not been a similar initiative to use nanoscience to explore the microbiome. If someone put $200 million on the table, people would work harder at overcoming those communication barriers, and we'd see significant and rapid advances.
Which leads me to another point. We need to create a data commons — a stronger, much more cohesive capacity to analyze multiple data streams. Just as we need to overcome communication problems between people, we also need to overcome communications between data so we can use everything we generate. That is, in itself, another grand challenge.
TKF: Another grand challenge?
J.G.: We have thousands of grand challenges. But it's a worthwhile effort to try and overcome them, to do nanoscience at the largest scales, because the largest scales achieve the greatest rewards.
Follow all of the Expert Voices issues and debates — and become part of the discussion — on Facebook, Twitter and Google+. The views expressed are those of the author and do not necessarily reflect the views of the publisher. This version of the article was originally published on Live Science.
Get the world’s most fascinating discoveries delivered straight to your inbox.