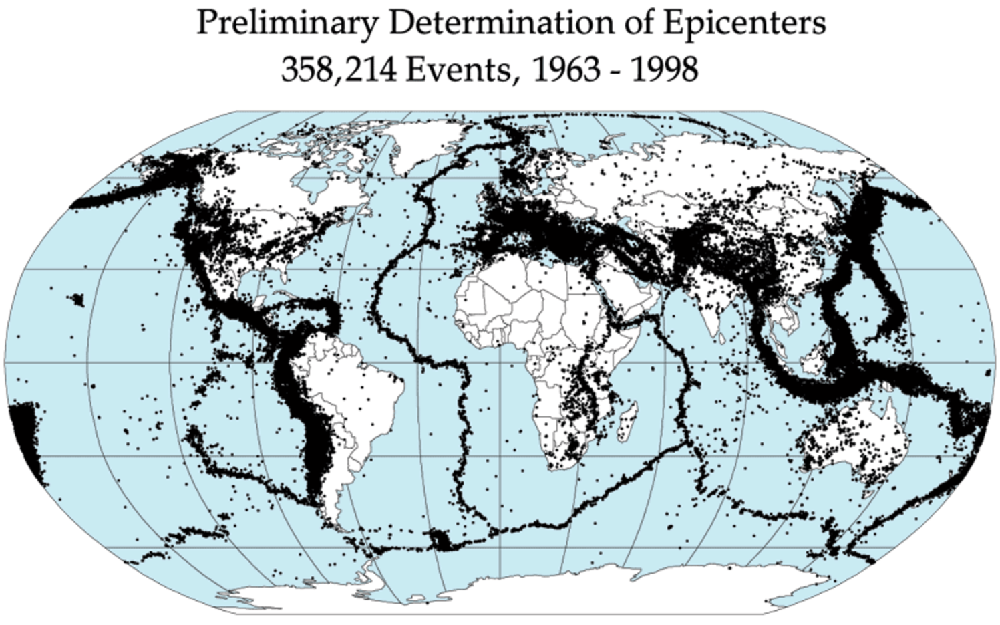
This article was originally published on The Conversation. The publication contributed this article to Live Science's Expert Voices: Op-Ed & Insights.
The past is never dead. It’s not even past. – William Faulkner
When disasters like the Nepal earthquake strike, seemingly out of the blue, one can’t help but feel anguish at the mismatch between the capacity of human memory and the tenacity of denial. The simple truth about great earthquakes, and the miserable cascade of events they often trigger, is this: if an earthquake has affected a region, recently or in historical records, then future earthquakes in that region are inevitable. But, if no damaging earthquake has happened in recent memory, it’s easy to ignore the need to prepare for a future event of uncertain magnitude and proximity. The earthquake cycle is long relative to the terms of a city council, a state legislature, and even a national government.
As a practicing seismologist, the political questions implicit in a discussion of how much risk a society is prepared to assume relative to the costs of mitigation are largely beyond my influence. On the other hand, seismologists like me can help address the question of where earthquakes have occurred in the past – and where they will occur again in the future.
We can estimate how large a magnitude earthquake can be expected in a given region. We can determine how different substrates – soils, sand, fill, bedrock – will affect ground shaking, and we can map the distribution of these foundational materials on a building-by-building scale, if necessary. We can assess the propensity for slope failure, which leads to landslides. And, for some regions, we can come up with ballpark estimates of the average time between large-magnitude earthquakes.
Even after a major quake, there’s much seismologists can learn that can hopefully help people prepare for the next one.
What do we want to know?
Scientists and policymakers ideally want to forecast the time, place and magnitude of a future earthquake. Knowing that information well in advance, we could issue a region-specific targeted alert, complete with estimates of expected shaking. Such knowledge would allow for the maximum safeguarding of populace and infrastructure. Perfect forecasting would also mean no disastrous failures-to-predict and no false alarms.
So what can seismologists do to get closer to this goal?
Sign up for the Live Science daily newsletter now
Get the world’s most fascinating discoveries delivered straight to your inbox.
It all comes down to plate tectonics
In seismology, our framework for understanding earthquakes begins with plate tectonics theory. The Earth’s surface is divided into around 12 major shell-like plates that move relative to one another. Earthquakes happen when the plates rub against each other or collide. We’ve observed that the vast majority of earthquakes occur within the wide (60-600 miles; 100-1,000 km) boundary zones at the edges of the slowly, continuously moving plates. Within these boundaries, plate motions are typically distributed on many active faults that sometimes slip – benignly! – slowly and continuously like the plates. But far more often the plate boundaries stick and are motionless for long periods before suddenly rupturing and producing catastrophic large-magnitude earthquakes.
Given the slow, steady motion of the plates, you might think that earthquakes on plate boundary faults would rupture periodically, say every few decades or centuries, when the stresses that build up on the faults due to the steady motions become greater than the frictional strength holding the fault still. Seismologists have been looking for such nicely behaved faults since the first precision-instrument recordings of earthquakes in 1889, but to no avail. We’ve yet to discover a predictable fault that has a quake right on schedule every 80 years, for example.
Recording at the surface for hints from within
We already know a lot about most major faults – where they are, their extents and depths, and at least their recent destructive histories. But there are many crucial things about these faults we don’t understand. The best-studied faults are basically covered with various instruments recording seismic phenomena, and I do mean covered: these observations are made only at the Earth’s surface, or very shallow depths.
We rely on seismic waves generated by earthquakes themselves to characterize the faults and their behavior. These waves of energy spread out from a rupturing fault and are recorded on seismometers and other geophysical instruments. Their characteristics, recognizable to seismologists, tell us about the type of earthquake rupture and the extent of the faulting. But, because these waves travel through complex materials on their way to the Earth’s surface, our ability to ‘see’ details of what happens at depth is inevitably compromised.
Seismic recordings have taught us that major fault zones are complex, typically involving multiple surfaces on which slip can and does occur. These surfaces are usually not continuous, but rather indicate that the major faults are segmented - planes of slightly different orientations juxtaposing potentially very different materials. Different segments of the fault zone can slip apparently independently, although they do influence each other.
Fault surfaces are rough, not smooth, and marked by asperities: sharp bumps, knobs and ridges on the walls of the fault that jab from one side into the other, creating locked points or patches. Stronger patches are more likely to remain locked until the steady plate motions build up enough to break them, while weaker patches slip slowly and steadily. Groundwater flow may both weaken fault rocks by dissolving minerals, or strengthen a patch of fault through precipitation of new minerals.
For every large-magnitude earthquake that occurs on a fault system, thousands or even tens of thousands of little earthquakes will occur. These low-magnitude events can be triggered by small changes in stress on the fault. For example, when seismic waves from a large-magnitude quake somewhere else in the world pass by segments of California’s San Andreas fault, the fault lights up with lots of little tremors. So we infer that many faults are near ‘criticality’ – at least some patches of the fault segments are ready to slip at any time, just waiting for a minuscule amount of stress to be applied.
If the faults are actually moving, just a little bit, essentially all the time, what has to happen for these little motions to coalesce into the big slip over a large area that would be a huge quake? Seismologists have been looking for consistently observed precursory phenomena – some change in fault behavior or structure that always, reliably, occurs before or even during the cascading of little earthquakes into a monster earthquake. So far, we haven’t found it.
Lots of science to be done after a big quake
Ironically, large-magnitude earthquakes like the Nepal event provide some of the most useful information for seismic hazard mitigation: the thousands of aftershocks in the following days and months occur all along the surface of the fault segments that ruptured. Seismologists usually rush to deploy many temporary seismic stations in the rupture region to record these aftershocks and then locate them with high precision – thus defining the fault’s slip surface accurately.
To do this well, we need to surround the rupture region with sensors that turn shaking due to seismic waves into electrical signals that are then recorded on a weather-proofed computer hard disk. The seismograms they record show the ground moving up and down and side-to-side systematically as the waves travel past the sensor.
The aftershock team’s work affords us an accurate measure of these parameters. Then we can make a firm estimate of the largest magnitude earthquake a particular cascading sequence of rupturing fault segments can produce. The upper magnitude limit for the region can then be used to estimate the maximum expected shaking, and, in combination with studies of substrate materials, expected hazard maps can be produced, building codes updated based on realistic expectations, and civil defense planning focused to mitigate specific disaster scenarios.
How to protect against future quake disasters?
The Nepal earthquake was long expected. A predecessor event in 1934 ruptured an even greater area, yielding a higher magnitude quake. And if earthquake preparedness there received less-than effective attention given this clear warning, imagine how much more difficult it is to motivate preparation in places that are susceptible to huge earthquakes, but whose most recent big quake occurred long before any of us were born, even before written history…. The past is never truly past, indeed!
Globally, we need a program of identification and characterization of potentially hazardous faults in urban areas. From those studies, site-specific expected seismic shaking maps can be developed and construction codes and engineering design specifications for infrastructure enacted, mitigating hazard to new and future construction.
Then urban political leaders and civil defense agencies must collaborate to lead local populations in an open and honest dialog to identify both irreplaceable cultural heritage, and also infrastructure that must survive natural disasters intact in order to prevent an earthquake from triggering a series of consequent catastrophes – fires, water and food shortages and disease outbreaks. These structures should be retrofitted to survive the predicted shaking from the maximum expected magnitude earthquake for the given area. A number of different mechanisms to pay for this costly preventive engineering are almost certainly needed, tailored to local conditions.
It’s clear the Earth has moved before and will move again, but will we move to do what’s necessary to mitigate preventable disasters?
Ray Russo is Associate Professor of Geophysics at University of Florida.
This article was originally published on The Conversation. Read the original article. Follow all of the Expert Voices issues and debates — and become part of the discussion — on Facebook, Twitter and Google +. The views expressed are those of the author and do not necessarily reflect the views of the publisher. This version of the article was originally published on Live Science.