
Volatile Sakurajima Volcano Is a Lightning Laboratory
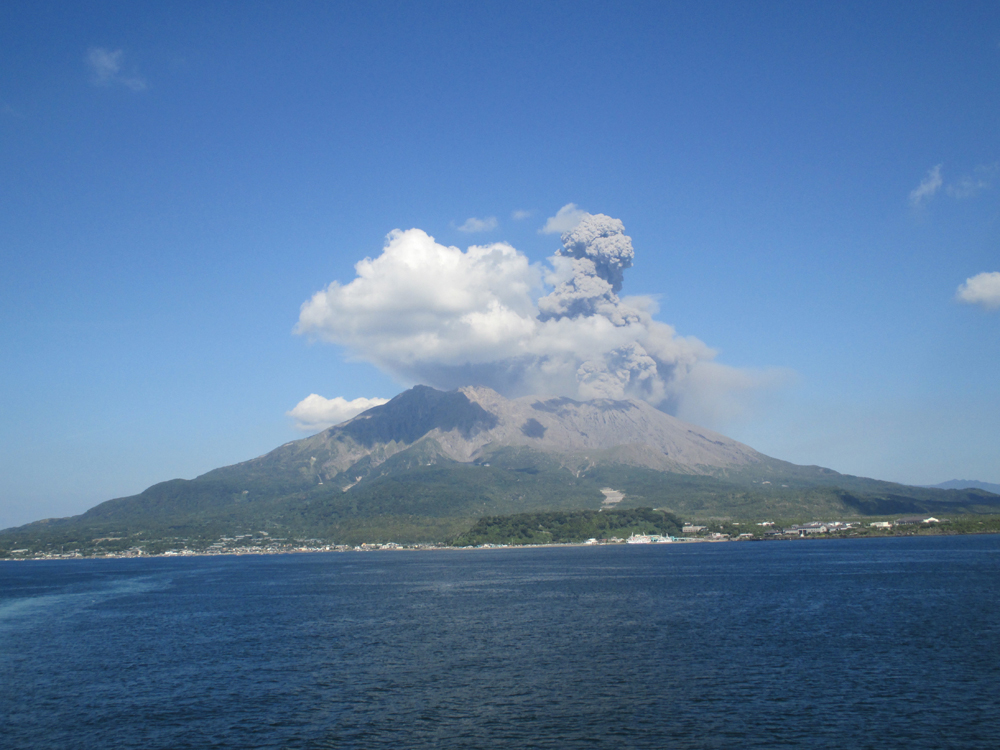
Jeffrey Johnson, associate professor of geosciences at Boise State University, contributed this article to Live Science's Expert Voices: Op-Ed & Insights.
Japan is a country of volcanoes, and Sakurajima is one of its most infamous. Its notoriety stems from its poor behavior in 1914, when powerful explosions and pyroclastic flows forced the evacuation of the small volcanic island. Shortly after the explosions stopped, extensive lava eruptions began. The amount of lava that erupted was enough to span Kagoshima Bay, connecting the volcano to Kyushu's mainland. For much of the next forty years, the volcano was relatively quiet.
But Sakurajima has been exploding intermittently since 1955. And although it no longer is an island, it is still nearly surrounded by water, and its 7,000 residents are exposed to volcanic hazards including ash fall, lahars, and the potential for lava bombs. Today, schoolchildren commute wearing hard hats just in case rocks start to rain down from the sky. Because ashfall often grays the landscape, everyone wears masks to avoid breathing in the tiny particles of volcanic glass. While downwind areas on the island are more vulnerable to the ashfall, no corner of the island remains completely unaffected, since any location is less than 4 miles from the exploding crater.
Although explosions are a worrisome inconvenience to the local population, the phenomenon draws volcano scientists to the region like moths to a flame: Sakurajima's reliable, frequent and powerful explosions provide researchers with an unparalleled laboratory for studying eruptions that are categorized as quintessentially "vulcanian."
On a typical day, a few vulcanian blasts can be expected to erupt out of the Showa Crater like canon shots. Ten seconds later, a concussion sound wave — often exceeding 100 pascals in pressure, akin to the sound pressure levels on an aircraft carrier deck — reaches the Kurokami Observatory 2 miles away. Most of this sound energy is subsonic, but if it were audible, it would be deafening: the equivalent of 140 decibels. To put it another way, it would exert a force of approximately 100 lbs. on a (well-sealed) window.
The blasts are accompanied by the blisteringly rapid explosion of gas and pyroclastic materials, which are composed of ash, rock bombs and refrigerator-size rocks. The materials erupting from the Showa Crater rim often exceed a velocity of 400 feet per second, and within moments, inertia carries the pyroclasts nearly 1,000 feet above the vent. A perceptive viewer might observe blinking sparks — lightning — in the growing column.
Observing volcano lightning processes in real time would be like trying to track camera flash bulbs at a sporting event. This is why Corrado Cimarelli of the Department of Earth and Environmental Sciences at Ludwig Maximilian University of Munich has been developing ingenious techniques to observe Sakurajima's eruption lightning events.
Sign up for the Live Science daily newsletter now
Get the world’s most fascinating discoveries delivered straight to your inbox.
Slowing down an eruption
Cimarelli and his co-authors recently published a study in Geophysical Research Letters entitled "Multiparametric observation of volcanic lightning: Sakurajima volcano, Japan." In this study, the researchers use high-speed cameras and magnetotelluric data (described below) to perceive the eruptive processes that are invisible and/or too fast for a human observer to track. While a consumer-grade video camera might capture image sequences 30 times each second, the high-resolution, high-speed cameras that Cimarelli uses capture images 100 times more rapidly.
During a single, high-speed image frame, exposed for 30 microseconds, the fastest ballistic particles travel barely more than an inch. This might appear unnecessarily fast for tracking pyroclastic trajectories, but it is an essential capability for gaining insight into the evolution of lightning, which "grows" at speeds of between 8 and 80 miles per second.
With the use of high-speed cameras, researchers have learned that lightning sparks propagate in a series of jerky advances known as stepped leaders, a process that is also seen in thunderheads. The stepped-leader response corresponds to the short circuiting of charged regions that have been separated either within a cloud, or between the cloud and ground. Once the connection is complete, current flows and heats the atmosphere, creating the visible pulse that observers recognize as lightning. [What Causes Eerie Volcanic Lightning?]
The sparks that are detected during volcanic lightning episodes at Sakurajima are generally small and measure between 30 and 600 feet — one or two orders of magnitude shorter than the lightning that appears during electrical storms.
The high-speed camera maps the distribution of sparks over time, but this information becomes much more valuable when it is complemented by magnetotelluric (MT) monitoring, which also detects sparks occurring within the opaque, center portion of the eruption column.
MT observations sample both electric- and magnetic-field variations from many miles away and at an incredible 65,000 times per second. Tiny magnetic-field fluctuations — about 1 part in 10,000 of Earth's ambient field — are well-recorded, and have revealed that Sakurajima volcano lightning carries up to 1,000 amperes of current. Using the MT technique with its valuable time-resolution capabilities, the research team can also count flashes, determine the direction of current flow for each flash and assess whether the lightning remains within the ash cloud (intracloud) or reaches the ground (cloud to ground).
Together, high-speed imagery of volcanic lightning and MT studies provide a fuller picture of the internal workings of a fiery, turbulent column of volcanic ash and gas.
Laboratory lightning
Although scientists' understanding of thunderstorm lightning is mature, they are only starting to build an understanding of volcano lightning. Based upon volcano lightning "mapping" studies conducted in Alaska, volcano lightning may be broadly grouped into categories that are described as "vent discharges," "near-vent lightning" or "plume lightning" depending upon where they are located within an eruption column.
The vent discharges at Sakurajima include sparks tens- to hundreds-of-meters long that occur near the mouth of the volcano. Here, small particles of ash erupt and are preferentially charged — that is, the larger particles becoming slightly more positive. And then, as particle sizes are sorted by air resistance within the ash cloud, they become physically separated. When the ash explodes upward, the smaller-size particles tend to slow down more quickly. This is when charge separation may occur, either due to fractocharging, as the pyroclastic material is violently ripped apart during eruption; or due to tribocharging, which is charge transfer through rubbing. This second mechanism is akin to the familiar static electricity that builds up when you rub a balloon on your hair.
Lightning is the response to the charge-separation process. Disequilibrium is remedied when the atmosphere short circuits and produces a spark — the lightning bolt. The current that is produced induces magnetic-field deflections that last for a millisecond, and occur with nanotesla intensity several miles away. The events are remotely recorded using MT methods.
Cimarelli believes that tribocharging plays an important role in the charge-separation process, because one of his earlier experiments involved the production of volcano lightning in the lab. The results were featured in a 2014 Geology paper, where he and colleagues ejected fine volcanic ash from a pressurized nozzle — a laboratory volcano vent — and generated lightning-like sparks a few inches in length. These sparks formed without obvious magma fragmentation and without the presence of ice — or graupel — which is the conventional charge-separation vehicle for lightning in a typical thunderstorm. [Electrifying Images of Volcano Lightning]
Why we care about volcano sparks
A wealth of volcano research has shown that eruption columns become statically charged due to ash separating in a plume. This is important, because near-vent volcanic lightning —including its intensity, frequency and character — is directly related to how much fine material is erupted. These discoveries are exciting, and suggest that soon we might be able to use lightning detection as a measure of how much ash is ejected during eruptions.
Other methods to calculate ash emission rates don't work very well. Satellite- and ground-based multispectral measurements can detect ash plumes, but don’t do a very good job when it comes to quantifying how much ash is in the plume, or to predicting the rate at which the ash is ejected. Cloud cover and darkness hinder both satellite- and ground-based visual observations of plumes, and deriving ash quantity is limited by our understanding of ash plume density.
Lightning detection, on the other hand, offers a means to potentially quantify ash discharges during inclement weather and at nighttime. Detectors can be located at safe distances, tens of miles from the vent, and the cloud does not impede the ability of MT sensors to "see" lightning.
Such detections are critical, as volcanic ash clouds are one of the principal hazards posed by eruptions. Even dilute amounts of ash that are ingested by a jet turbine can incapacitate the engine, causing it to fail catastrophically. This potential hazard was brought to the attention of the general public by Iceland's 2010 Eyjafjallajökull Volcano eruption, which spewed ash across the air corridors of Europe. The eruption grounded more than 100,000 flights over the course of a week, affecting 10,000,000 travelers, and causing billions of dollars in losses.
Given the economic impact of ashy eruptions, the next generation of comprehensive eruption monitoring will focus on ash quantification and will likely use lightning ash detectors as a primary instrument. Sakurajima, a laboratory volcano in Southern Japan, is facilitating the development of this tool.
Follow all of the Expert Voices issues and debates — and become part of the discussion — on Facebook, Twitter and Google+. The views expressed are those of the author and do not necessarily reflect the views of the publisher. This version of the article was originally published on Live Science.