'A remarkable conspiracy': Why is matter neutral? Physicist Frank Close explores the mystery in a new book
Frank Close tells us about the history of particle physics, and what it means that charge in our universe's matter is so closely balanced.
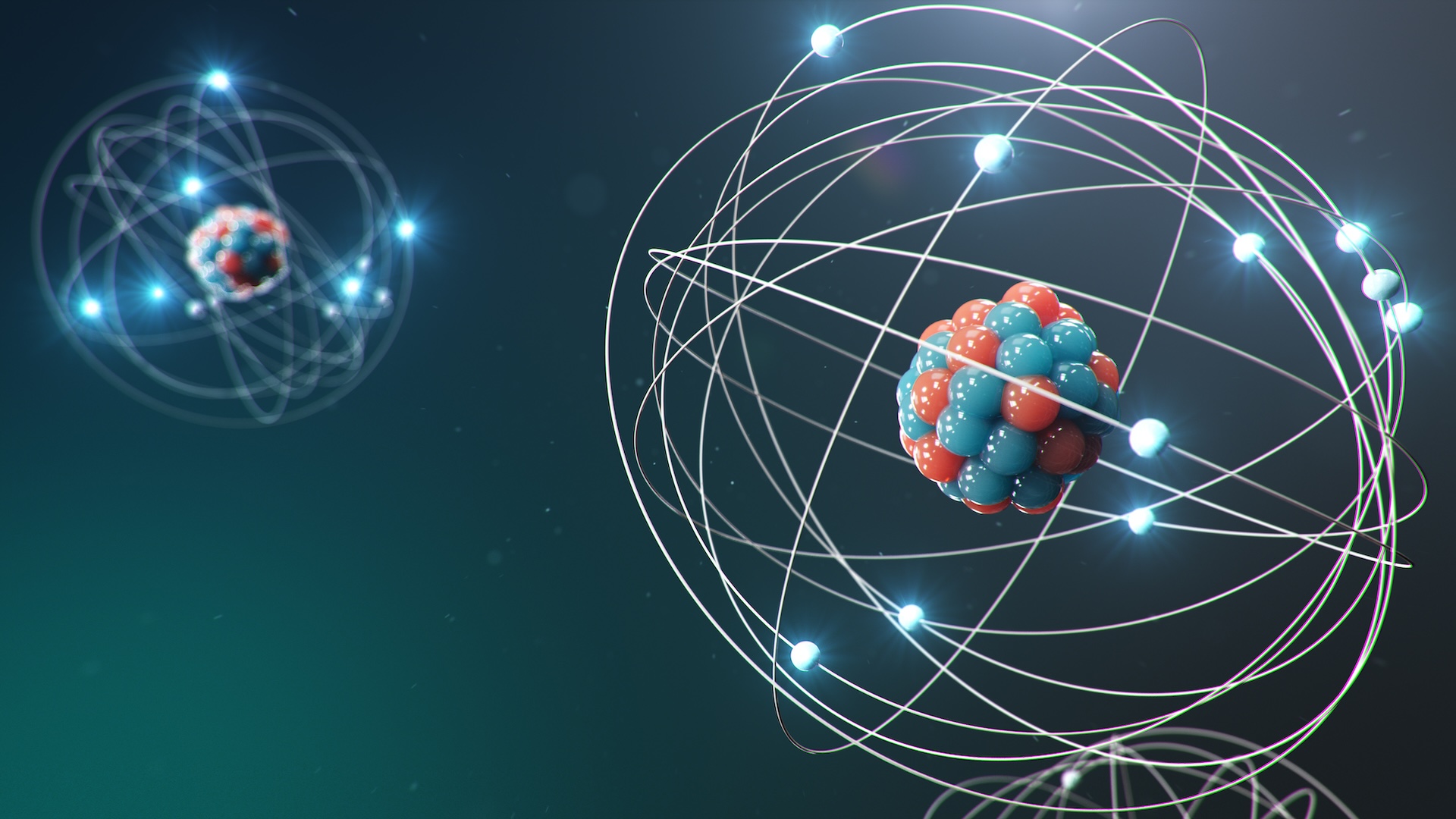
Since the discovery of the proton and the electron in the 20th century, a mystery persists at the core of the atom: Despite belonging to completely different particle families and being radically different in size, the charges of these two particles completely balance each other out — enabling a universe where gravity dominates. But why?
To explore the clues, Live Science sat down with Frank Close, an author and emeritus professor of particle physics at Oxford University, to discuss his new book "Charge" (Oxford University Press, 2024). In it, Close traces out the conundrum through a concise history of particle physics, including the strong, weak and electromagnetic forces that operate over short distances, the discovery of the Higgs boson, and the hints of a yet-to-be-discovered grand unified theory.
Ben Turner: Your book provides a fascinating summary of the current state of particle physics, and the remaining mysteries in it — most importantly the electrical neutrality of matter. What motivated you to write it? And why now?
Frank Close: It's been a puzzle that's been with me for a long time.
Why is it that, every breath you take, your hair doesn't stand on end, given that you're breathing in a billion billion billion atoms of oxygen and nitrogen in the air, each of which has got all this electricity in it? The negative charge on the electrons of all these atoms is triflingly small, but there are so many of them together that a single breath is like breathing in roughly 15,000 coulombs — that's enough to spark 1,000 bolts of lightning.
The answer is that the atom is electrically neutral. The negative charge of the electron outside is precisely balanced by the positive charge of the nucleus in the middle.
It's one of the unanswered questions in science, and it strikes me, perhaps, as the most immediate one. You don't have to have a huge theoretical background to notice it.
Sign up for the Live Science daily newsletter now
Get the world’s most fascinating discoveries delivered straight to your inbox.
BT: "Why" is always one of the more fraught questions to ask in physics, but I'll do it. Why might charges be perfectly balanced?
FC: It's a solid question you might ask a really smart PhD student doing their exam. You know that you don't know the answer and they don't either, but it might terrify them for a moment.
It's the puzzle at the heart of the book. If we had this discussion a century ago, the only particles that were known were the negatively charged electrons and the positively charged protons. I would probably have told you that I don't know quite what charge is, but it is something that you can take away or add and that in the case of the electron it's had it removed and the proton has had it added.
The problem is that we now know much more. In the 1960s we discovered that the proton has a structure, it's made up of things called quarks. Up quarks have two thirds of positive charge, and down quarks negative charge of one third. The simplest way to make a proton is out of three quarks — two up quarks and one down. If you want to make a [neutrally charged] neutron you use two down quarks and one up.
So the equality between the plus one charge of the proton and the minus one charge of the electron is a remarkable conspiracy. Is that a coincidence? I don't believe in coincidences. But it shows the conundrum is not simply a matter of painting charge onto the proton and removing it from the electron.
BT: I'm asking why again, but why do quarks clump in threes? What causes it? And what's its relationship to electrical charge?
FC: Quarks carry another sort of charge, which we call color. This color charge occurs in three different varieties: red, blue and green. They're not real colors, but there are three of them and they follow the same rules as electrostatic charges — with like charges repelling and unlike ones attracting. So it's this 'threeness' of the color charges that help them clump powerfully to form the proton. The fact that each of them, on average, carries one-third electrical charge is what makes the conspiracy work.
There's something tantalizing going on. Color charges follow the same rules of attraction and repulsion as electric charges do. You feel that, on some level, these things are profoundly related, even though you don't quite know how. You feel like you're on the edge of something. If only we could just see a little bit more clearly, it would all fall into place.
BT: And that's possibly that they're all connected, or branchings of the same thing at different energies, a so-called grand unified theory of everything?
FC: They must be fossil relics of something much more significant, powerful and unified, from which the idea of a unified theory begins to emerge. We've clearly stumbled upon something here: the 'threeness' of these [short range] forces as we know them in the cold universe today.
We know from very precise experiments over the last 30 or 40 years that, as you go to higher energies at the Large Hadron Collider, that the relative strength of these [fundamental] forces does change slightly. If you extrapolate that, it means that at some unimaginably high energy these three forces [the strong force via color charge, the electromagnetic force via electrostatic charge, and the weak force via the W and Z bosons] have roughly the same strength.
BT: You used the word tantalizing to describe these hints. If that's the case, how close might we be to finding a grand unified theory? When did physicists begin to chase this idea?
FC: Mathematically, there's no difficulty in creating grand unified theories.The frustration of being a theoretical physicist is that experiment keeps showing you that you're wrong.
For 2,000 years we've been searching for what matter is made of, and we've found deeper layers of structure by going to higher and higher energies. Around 1970, the idea emerged that at extremely high energies things might be simple and that the early universe was also very hot.
At CERN, we started doing experiments that initially annihilated electrons and their antimatter counterparts, positrons, so that their light-speed kinetic energy was converted instantly into a flash of pure energy. In a very small region, for a brief moment, you've got the sort of energy density that would have been present in the universe about a billionth of a second after the Big Bang.
Observing what emerged from that 'mini bang', we began to understand not so much what matter is made of today, but how matter came to be in the first place. That began a psychological transition from particle physics to experimental cosmology — it was no longer just stamp collecting, we were replicating the aftermath of creation.
BT: At the present time, particle physics is slowly moving into higher energies and cosmology is getting a lot better at looking back into the hotter, earlier years of the universe — peering into the primordial particle collider. What are the big open questions that remain?
FC: I think probably the first one is to go back 10 years to the discovery of the Higgs boson. What does its discovery actually mean and where should we go from here?
There's something very profound about the Higgs boson. It confirms that, if you took everything away — all the particles, all the sources of charge and gravity and everything else in the universe — there'd still be something left. Some weird essence that we call the Higgs field. What it is, we have no clue, but it's there.
We and everything are immersed in the Higgs field, and we need it just like a fish needs water. We know it's there because (much like an electromagnetic field does with photons) if you add a bit of energy to the Higgs field it will bubble up as Higgs bosons. This means that, in the heat of the Big Bang, Higgs bosons were everywhere.
It's as if a very clever goldfish had discovered a molecule of H2O. It now knows it's immersed in water, but what it really wants to know is what water is like: "What's water? What's ice? What's steam?"
In a similar way, we want to know if the Higgs field has different phases and how it operates. At the moment we can produce one Higgs boson periodically, but could we produce two at the same time in a single collision and see how they interact? That's the immediate goal at CERN, and in the next decade or so I'm sure that answer will begin to emerge.
Editor's note: This interview has been condensed and edited for clarity.
CHARGE: Why Does Gravity Rule?
$21.99 on Amazon
If you enjoyed this interview with Frank Close, you can read more about the mysteries of electric and magnetic forces in his new book, "Charge."
—Discover where magnetism comes from in this excerpt from the book
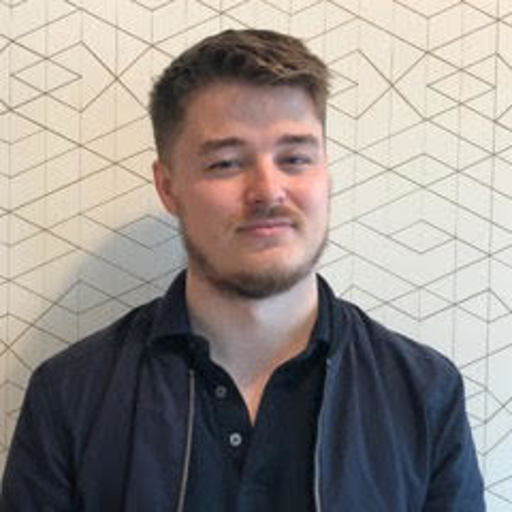
Ben Turner is a U.K. based staff writer at Live Science. He covers physics and astronomy, among other topics like tech and climate change. He graduated from University College London with a degree in particle physics before training as a journalist. When he's not writing, Ben enjoys reading literature, playing the guitar and embarrassing himself with chess.