Take a tour of the synchrotron, where electrons reach near light-speed
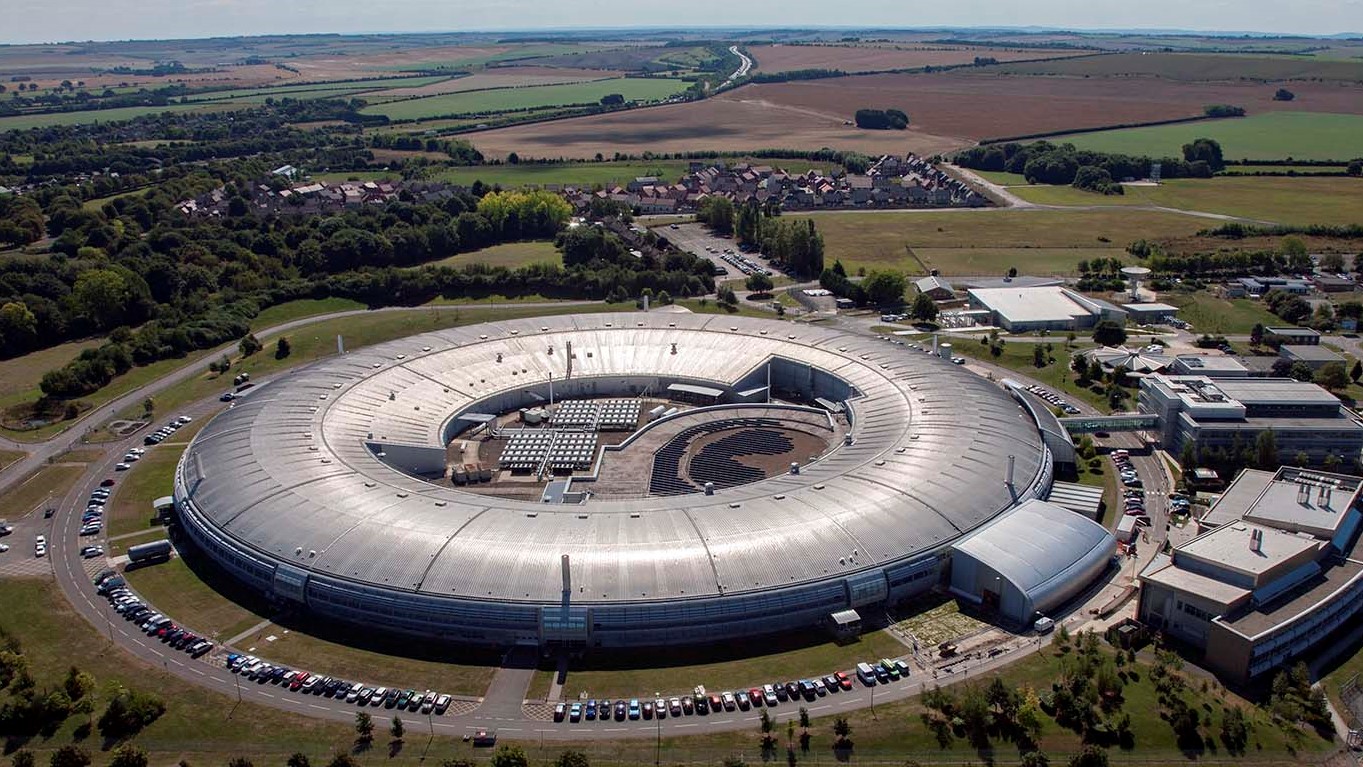
Electromagnetic (EM) radiation is incredibly useful. It enables us to transmit music wirelessly over large distances, cook food in the microwave and see the world in vivid detail. However, now more than ever, electromagnetic radiation is also crucial in studying the physical, environmental and biological phenomena that are leading to real breakthroughs for people.
From the creation of new medical drugs and vaccines, to the testing of revolutionary artificial organs, to discoveries that allow diseases to be prevented, the harnessing of EM radiation on a large scale is expanding horizons in the scientific world.
In the U.K., that revolution is happening at the Diamond Light Source national synchrotron facility in Oxfordshire, a high-tech particle accelerator that generates vast quantities of EM radiation in the form of synchrotron light. Let's take a trip to this cutting-edge science site to see what working there is like on an average day and what groundbreaking experiments are currently being investigated.
Exploring the synchrotron
A synchrotron is a large, complex system of machines that generates electrons, accelerates those electrons to near light-speed and then deposits them in a large storage ring. The high-energy electrons then fly around the ring circuit continuously until they are manipulated to generate very high-intensity X-ray light; these are electrons with around 3 gigaelectronvolts (GeV), a GeV being a unit of energy equal to a billion electron volts. This is the light that scientists can utilize in their experiments.
This article is brought to you by How It Works.
How It Works is the action-packed magazine that's bursting with exciting information about the latest advances in science and technology, featuring everything you need to know about how the world around you — and the universe — works.
Guenther Rehm is head of the Diamond synchrotron's beamline diagnostics group, which is responsible for ensuring that when visiting scientists need X-ray light, they are able to get it. Rehm's office in Diamond House is a sleek, glass-walled complex where the majority of the facility's staff are based. To get to the synchrotron facility, you have to then cross a security-controlled bridge.
Once there, you would see four main parts, the first of which is an electron gun. Sitting at the heart of the facility, this gun is responsible for generating electrons by heating a high-voltage cathode in a vacuum, then forcing them to bunch up together and compress into compact groups; this is achieved by passing the beam of electrons through a cavity where an alternating electric field is active.
From the bunching cavity, a beam of compressed groups of electrons passes into a linear accelerator. This part of the synchrotron uses a series of electric fields to force the compressed electron bunches to accelerate to close to the speed of light and up to a charge level of 100 megaelectronvolts (MeV). From here, the sped-up bunches of electrons are injected into the booster synchrotron.
Sign up for the Live Science daily newsletter now
Get the world’s most fascinating discoveries delivered straight to your inbox.
The booster synchrotron sits just off the linear accelerator. It is a 518-foot (158 meters), O-shaped stainless-steel tube vacuum surrounded by magnets that sits within the synchrotron's storage ring and other facilities. This smaller synchrotron receives the electrons, and then — with the help of 36 dipole magnets — bends them around the vacuum circuit while they are accelerated further up to the necessary extraction energy of 3 GeV. Traveling at almost the speed of light and carrying an insane amount of energy, the electron bunches are lastly injected into the synchrotron's storage ring.
The storage ring is similar in both build and purpose to the booster ring, but on a far larger scale: The ring, which is a 48-sided polygon, spans more than 1,800 feet (560 m). Luckily, the electrons have so much energy they can whiz the entire course in 2 millionths of a second; for comparison, that's 7.5 times around Earth's equator in just 1 second. To keep things moving, the giant ring consists of a vacuum in which the charged electrons travel, and a series of magnets, including dipole-bending magnets to maneuver the beam around the circuit, quadrupole magnets and sextupole magnets to ensure accurate beam focus and position. The ring also holds special magnets called insertion devices (IDs) to manipulate the electrons for synchrotron light production.
The IDs are the real stars of the synchrotron, capable of getting the passing electrons to oscillate around through the straight sections of the ring. As a result, super-powerful X-rays are produced. Because these IDs are so critical, they are always placed ahead of any beamline — offshoots from the ring where experiments take place. The electrons enter the device, oscillate and create X-rays. While the electrons are flung farther down the storage ring by dipole magnets, photons continue straight down the beamline for use in experiments.
Staying in control
Next, you would arrive at beamline central control. A large, spacious room overlooking approximately a third of the expanding facility, the area is filled with a main bank of monitors; there, two members of the diagnostics team run the computer systems. Rehm explained that the day-to-day operation of the synchrotron is heavily automated, hence the minimal staffing. However, due to the incredible complexity of the systems involved in creating and maintaining high-energy electron beams, actual humans must monitor the status of the complex.
At all times, a software program called EPICS: Experimental Physics and Industrial Control System monitors the beam in the storage ring. This allows the invisible beam's properties to be visualized via a variety of sensors, monitors and cameras within the ring.
Rehm demonstrated that in a period of just over 10 minutes, the bunched electrons in the storage ring suffer inevitable loss. This is due to collisions and residual gas molecules, as well as energy loss through the generation of synchrotron light by the insertion devices and bending by the dipole magnets. To maintain optimal beam stability and synchrotron light quality, the charge is automatically boosted periodically. Watching a live graph in EPICS, you could see how the overall charge level drops within the ring and then, precisely after 10 minutes, returns back to its start level.
Not only is this boost automatic, but the system can actually target the parts of the beam from which the electrons have been lost; this makes for an even, stable distribution of energy around the ring for light generation at all times, Rehm said. This system is truly amazing, capable of injecting additional electrons into the depleted electron bunches smoothly as they fly around the storage ring at almost light-speed.
Looking down the beamline
Moving to the heart of the facility, you would enter the cavernous main room of the synchrotron. When standing on an elevated gantry bridge, stretching out to both sides, you would see the curved expanses and many of the synchrotron's individual beamlines, branching off from a concrete ring. This is the facility's storage ring, which is encased within thick, radiation-blocking concrete shielding. On top of the concrete ring is a yellow line that identifies the actual path of the electron beam inside. According to a tour guide at the facility, a person could lie on top of the concrete for an entire year and receive a radiation increase of only approximately 50% over that from standard background radiation. Simply put, very little radiation escapes the ring.
Sandwiched between two beamlines is a small, black room. Upon entering, you would find a large table stuffed with machines, pipes, optics and cabling. Behind this, a small hole is cut into the wall. This is the optics diagnostics cabin, and it allows the support scientists to explore the temporal structure of the stored electron beam, revealing its fill pattern — how much charge is in each of the electron bunches.
Handling the light
Knowing how the synchrotron works is one thing, but what can it do in the real world? Enter Nick Terrill, the principal beamline scientist for the small angle scattering and diffraction beamline (also called I22). Among many other examples, Terrill describes how a team recently used I22 to test new polymer-material artificial heart valves. The team built a tiny device to stretch the valve to reproduce the effects of a heartbeat and then used the synchrotron’s high-energy X-ray light source to image the internal structure of the polymer valve in continuous resolution over a long period. These sorts of polymer valves will soon be a common replacement for problematic mechanical and animal implant valves.
After a short walk around the synchrotron's outer walkway to beamline I24, you would come across the microfocus macromolecular crystallography station. I24 is staffed by Diamond's senior support scientist Danny Axford, who explained how the team is working on membrane proteins, exploring their structures — something that is important in the creation of new drugs, among other applications.
Inside I24's experiment room, you would see liquid-nitrogen storage tanks, imaging sensor, robotic arm, synchrotron light-focus optic and a sample array. With the array, scientists can image rows of crystals at room temperature. This is incredibly useful, as heat from the imaging process damages crystals, so capturing their structure quickly is crucial — hence why many samples are cryogenically cooled.
The next port of call is the small molecule single crystal diffraction beamline (I19), where a variety of crystallized samples are being analyzed through diffraction techniques, with samples for projects involving everything from cancer to hydrogen storage. Next door in I20 is an impressive, versatile X-ray absorption spectroscopy beamline, run by principal beamline scientist Sofia Diaz-Moreno.
This beamline, which is much larger than any of the others, has two experiment hutches that share the line to enable different types of spectroscopy analysis. This type of analysis can image the chemical components in catalysts — even in very low concentrations. This ability to image reaction processes at an atomic level and at microsecond time scales is truly mind-blowing, and it is allowing scientists to understand things such as catalysts, metalloproteins — metal ion-containing proteins — and toxic materials like never before.
Racing the electron beam
There's one final stop: a stroll on the roof of the storage ring. Ascending back up to the first floor from beamline level and crossing the metal gantry toward the center of the facility, you would break off and step directly on top of the concrete roof of the storage ring before following the yellow beamline marker around the facility.
It would take nearly 10 minutes to make a full circuit around the ring — much slower than the two-millionths of a second needed for the hyper-charged electrons to whiz around the ring.
How It Works has a special formula for making learning fun by answering questions on science, space, history, technology, transport and the environment with engaging articles, in-depth special features, global science news, and topical interviews. With impressive cutaway illustrations that show how things function, and mindblowing photography of the planet’s most inspiring spectacles, How It Works represents the pinnacle of engaging, factual fun for a mainstream audience keen to keep up with the latest tech and the most impressive phenomena on the planet and beyond. Written and presented in a style that makes even the most complex subjects interesting and easy to understand, How It Works is enjoyed by readers of all ages.
Get fantastic offers by subscribing to the digital and/or print edition now. Subscribers get 13 issues per year!